Saturday, August 30, 2014
Active galaxies and supermassive black hole jets
All black holes gravitationally attract any nearby matter, because of their high mass, which is generally ≥ 1 M⊙ even for comparatively tiny stellar mass black holes. Such matter does not necessarily fall directly into the black hole, but instead can go into orbit around the black hole. If there is enough matter close to the black hole, and if it is pulled in rapidly enough, the results can be a spectacular light show, such as one might see (if one could see simultaneously at all wavelengths from very high radio frequencies to X-rays) in the Centaurus A galaxy, about 13 million light years away:
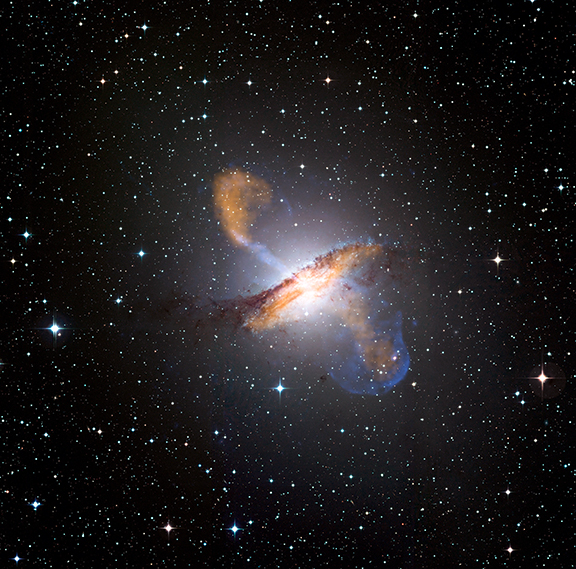
This is a view of the whole galaxy – you can see that the central area, which contains the black hole, is unusually bright, and there are jets extending more than the radius of the galaxy itself in both directions perpendicular to the galactic plane. Centaurus A is an example of an "active galaxy", and it shows the impressive effects produced by the central black hole of such an object. (For more about Centaurus A and this image, see here, here. Another image: here.)
The innermost region of an active galaxy, which is the interesting part, is called an "active galactic nucleus" (AGN). This is a general term for a number of puzzling astronomical objects that were noticed at first on account of their unusually vigorous output of energy, but whose similarities were not immediately recognized. AGNs were eventually deduced to be (in almost all cases) just relatively ordinary galaxies with massive central black holes that appear to be responsible for liberating at least as much energy as all the stars in the remainder of the galaxy.
Although most galaxies seem to have a supermassive black hole in their center, behavior of AGNs is rather unusual, and AGNs are somewhat rare in the nearby universe, but more common at large distances – hence at an earlier time in the universe. AGN behavior requires not only a supermassive black hole, but also a substantial amount of surrounding interstellar gas that fuels their energy output. AGNs are rather profligate in using their fuel, so presumably most of the available interstellar gas close to a central black hole is consumed over a relatively short period of time (compared to the age of the universe). Consequently, in most nearby galaxies the fuel was used up long ago.
The Milky Way has a relatively small central black hole associated with the radio source named Sagittarius A* (Sgr A*). The black hole has a mass of ~4×106 M⊙. Sgr A* is not nearly luminous enough to be considered an AGN, so evidently either there is not now enough nearby interstellar gas, or perhaps the black hole is just too small to have ever attracted enough.
How luminous does a galactic nucleus need to be in order to qualify as an AGN? Its really a question of how bright the nucleus is compared to the rest of the galaxy. Messier 77, also known as NGC 1068, is the first galaxy now considered to have an AGN that came to special attention. In 1908 E. A. Fath obtained its spectrum and found it had unusually strong emission lines. (This was at a time when it was still assumed that nebulae were simply fuzzy objects inside our own galaxy.) V. N. Slipher later obtained a better spectrum and noted that the width of the lines implied high velocities – hundreds of kilometers per second. NGC 1068 is fairly nearby – 47 million light years away – and rather large, with a diameter of 170,000 light years (compared to the Milky Ways diameter of 100,000 light years). NGC 1068 is still under active study at this time – see here.
Finally in 1943 Carl Seyfert recognized that NGC 1068 was similar to a number of other galaxies that formed a distinct class, based on the nature of their spectra and because their innermost regions were as bright as the entire rest of the galaxy. This concentration of luminosity in the center was not only exceptional, but it was quite unlikely to be physically possible for a sufficient number of stars to be located in such a small volume of space. Naturally, galaxies of this sort became known as Seyfert galaxies.
Other peculiarities of Seyfert galaxies were eventually recognized as well. For example, their spectra contain broad, strong emission lines of hydrogen, helium, nitrogen, and oxygen. This in turn implied that the emitting material had to be in rapid motion in order to produce Doppler broadening of the emission lines. And this in turn implied that a large amount of mass needed to be concentrated in a small volume to account for such high velocities. The characteristics of high central luminosity and broad emission lines tended to occur together enough to justify recognizing Seyfert galaxies as a distinct class, which made up about 1% of nearby spiral galaxies.
About 15 years after Seyfert galaxies were discovered, another peculiar type of astrophysical object was noticed – quasars, or, as they were sometimes known, "quasi-stellar-objects". These came first to attention as strong sources of radio emission, in early radio telescope surveys, such as the original Third Cambridge Catalogue of Radio Sources. The strong radio signal was somewhat mysterious, since electromagnetic radiation at radio frequencies (up to 100 GHz at the high end) is normally emitted only by rather cold matter (under about 2 degrees above absolute zero).
Many of these sources ("radio galaxies") were eventually identified with optically visible objects, many of which had already been cataloged as unremarkable stars. But when they came under attention as radio sources and their optical spectra were obtained, their high redshift implied that they needed to have an astonishingly high intrinsic luminosity – too luminous to be explainable simply as very large galaxies containing even the brightest possible stars.
Phenomena that are seemingly impossible to explain in terms of familiar examples tend to draw a lot of attention – and this is true, in some respects, of quasars even today. A lot of the mystery is now explainable in terms of active galaxies that are powered by supermassive black holes, and well get more into that in a little bit. But there are also some AGN features, such as the jets that a few AGNs expel at relativistic velocities, which are still not well understood.
Although there was still controversy at the time whether redshift could be used as a reliable gauge of distance, if the conventional redshift interpretation (Hubble expansion) was assumed, then quasars would need to be (what was considered at the time, ca. 1960) extremely distant. One of the earliest-recognized quasars, 3C 273, which is the quasar with the largest apparent magnitude and is visible through amateur telescopes, has z=0.158, corresponding to an optical distance of ~2.4 billion light years. 3C 273 was therefore intrinsically far brighter than any star, about 100 times as bright as an entire spiral galaxy. 3C 273 is sometimes considered to be the nearest unambiguous quasar. So bright quasars are absent from the local universe, although there are ambiguous cases as close as ~800 million light years (z=0.06).
Its now pretty clear that all quasars are extremely luminous active galaxies, but when 3C 273 and similar objects were first discovered they were point-like objects without the visible appearance of a galaxy. After all, the rest of the galaxy of which they were a part was only 1/100 as bright as the nucleus. So the objects were referred to as "quasi-stellar objects" (QSOs), or quasars for short. (Sometimes the term QSO was reserved for the minority of such objects that did not have appreciable radio emissions, while "quasar" meant a QSO with a strong radio signal.)
Its also clear now that the distinction between Seyfert galaxies and quasars is rather arbitrary – the brightest Seyferts have characteristics much like the least bright quasars. Further, there are a small number of characteristics which may or may not be present in either class. Some of the "optional" features that may be present include X-ray emissions, narrow (optical or ultraviolet) emission lines in the spectrum, broad (optical or ultraviolet) emission lines, strong radio emissions, and evidence of relativistic jets of matter (such as seen in Centaurus A, above).
The last two of these features – radio emissions and jets – almost always are either present or absent together. Naturally, the jets are expected to account for the radio emissions, and there are very reasonable models that explain the connection. However, only about 10% of all AGNs, when Seyfert galaxies are included, have the jets and strong radio emissions, so whatever physical process is responsible for AGN behavior doesnt automatically produce relativistic jets as well.
Indeed, the actual physical process responsible for jets is not well understood, and its one of the more mysterious phenomena in astrophysics. The research to be discussed here is a contribution towards elucidation of the mystery.
Physical models
But first, lets quickly review the physical model that now has consensus support to account for AGNs in general. The main characteristic, which was at first the most puzzling, is the enormous quantity of energy emitted in a very small volume of space. How small a volume? One indication is that the energy output can vary over periods of just a few days. Consequently, the diameter of the source must be only a few light days – about 10 times the diameter of Plutos orbit. And yet the source may produce energy ranging from 1 to 100 times as much as an entire good-size galaxy containing hundreds of billions of stars. A little breath-taking, when you think about it.
So where does all this energy comes from? Its not thermonuclear energy thats produced by fusion the way that stars do. The material around even the largest black hole is not dense enough. Thats basically because of whats known as the Eddington limit. Any time a sufficiently large mass of gas is collapsing under the force of gravity, the potential energy of the gas is converted to electromagnetic radiation. The energy released as radiation increases to the point where the outward radiation pressure equals the pressure due to gravity, stopping the collapse. This is what prevents very large stars – more than about 100 M⊙ – forming out of a large mass of gas. The same process also limits the rate at which gas can collapse around a black hole.
However, in the case of a supermassive black hole it is exactly this conversion of potential energy into radiation that supplies the enormous output of energy found in AGNs. Lets look at a simple calculation to show just how effectively a very large compact gravitating object can release energy.
Consider a supermassive black hole whose mass MBH is 109 M⊙. Since M⊙=1.99×1030 kg, we have MBH=1.99×1039 kg. The black hole is surrounded by an event horizon – the boundary from the inside of which neither matter nor radiation can escape. In the simple case of a non-rotating black hole the event horizon is a sphere whose radius is the Schwarzschild radius, which is rs=2GMBH/c2, where G=6.67×10-11 m3 kg-1 sec-2 is the gravitational constant and c=3×108 m/sec is the speed of light. Plugging things into the formula gives rs=2.96×1012 m. Thats very close to the radius of the orbit of Uranus.
Next lets ask how fast an object or particle in orbit around a supermassive black hole might be moving. There is a very simple formula for orbital velocity: v≅(GM/r)½, where M is the mass of the central object, and r is the radius of the orbit. Thats an approximation, since it makes some assumptions – the orbit is nearly circular, and the mass of the orbiting object is much less than M – reasonable for the sake of discussion. Squaring both sides and rearranging: r=GM/v2. We could plug in various values and see what we get, but suppose we want to know r in some reasonable units, such as the black hole Schwarzschild radius rs=2GMBH/c2. Then using plausible values for v, say v=c/10. Thats "small" enough that relativistic effects are minor: the Lorentz factor γ=(1-(v/c)2)-½≅1.005. The result is that r/rs=100GMBH/2GMBH=50.
So an object or particle in orbit around a black hole at a distance of 50 rs is moving at 1/10th of the speed of light. Notice that this isnt dependent on the black holes mass – its true for any black hole. (It doesnt apply to objects that arent black holes, since their Schwarzschild radius is very small, much smaller than the size of the object.) This calculation isnt necessarily realistic physically, since it neglects a number of other considerations, for example gas viscosity and turbulence. But it shows that black holes are nothing to be trifled with – they can have rather sizable physical effects.
In fact, although c/10 is not quite a relativistic velocity, its still rather sprightly. For instance, at that rate one could get from the Sun to the Earth in an hour and 23 minutes – faster than the commute into a big city in bad traffic. Its also a velocity that gives even something as small as a proton quite a bit of kinetic energy. Lets compute it. The proton mass mp≅1.67×10-27 kg. Kinetic energy E=mv2/2 = (1.67×10-27)(3×108/10)2/2 ≅ 7.5×10-13 kg m2 sec-2 = 7.5×10-6 ergs. Since one erg is 6.2415×1011 eV (electron volts), the kinetic energy of a proton moving at 1/10th the speed of light is about 4.68×106 eV = 4.68 MeV.
Thats not chicken feed – its well within the gamma ray range (100 keV to several 10s of GeV). What this means is that in any collision between protons moving this fast, its no sweat at all to give off gamma-ray photons, or photons of any other form of electromagnetic energy. And this is how black holes of any size, from stellar mass up to the supermassive kind, can convert a substantial fraction of the mass-energy of matter that falls in sufficiently close to electromagnetic radiation.
Given all this, the questions that occupy astrophysicists interested in supermassive black holes, AGNs, quasars, and the like include: Whats the exact physical configuration in which the energy is released? What processes bring about the energy release? How do these physical details explain observable effects, such as total energy output, emission lines, relativistic jets, and so forth?
Astrophysicists have been working on these questions for at least 50 years, since the first quasars were discovers, and a consensus has emerged about many of the physical details.
The main feature that all AGNs have (at least in the standard model) is a substantial accretion disk of matter orbiting around them. In many cases that have been studied in detail, theres a lot of evidence for such disks, besides the powerful emission of electromagnetic energy at frequencies from far infrared to far ultraviolet. As the name implies, the disks are flat and relatively thin. The inner and outer radii of the disks vary from case to case, but since there are minor fluctuations of output over periods of days, the inner radius must be on the order of at most a few light-days, around 1014 m, or 1000 times the size of the Earths orbit.
Detailed analysis of the physics indicates that the innermost part of the disk should be the hottest, with the temperature gradually tapering off toward the outside. Since the emission is thermal ("black body"), the relation between temperature and wavelength is given by Wiens law: T=b/λmax, where b≅2.9×10-3 m-K. λmax is the wavelength at which intensity per unit wavelength is maximized. Thus peak temperatures may range from 300,000 K when λmax=10-8 m (ultraviolet) down to 300K when λmax=10-5 m (infrared) – possibly even more at the high end. Higher energies and temperatures actually occur with stellar mass black holes instead of the much larger supermassive ones, because the maximum rate at which matter can accrete (the Eddington limit) is higher for smaller black holes.
As explained above, the energy to heat disk material to such temperatures comes ultimately from gravitational potential energy as matter falls inward and gains kinetic energy, which manifests as heat and ultimately electromagnetic radiation. The efficiency of this conversion of matter into EM energy can actually reach about 10%, which is a lot higher than nuclear fusion, for which the efficiency is only about 0.7%.
The net result is that a certain fraction of matter (mostly diffuse hydrogen and helium gas) in the vicinity of a black hole is converted to electromagnetic energy. This process can go on for a long time (perhaps hundreds of millions of years) until the matter is mostly used up or falls into the black hole itself. Calculations have verified that this process is entirely adequate to account for the observed luminosity of AGNs.
Eventually there is not enough matter sufficiently close to the black hole to be sucked in, and the process stops. This is why most quasars are observed only at great distances – more than a billion light years – because they no longer have the means to sustain the extremely high luminosity. It could be that all or most galaxies go through a quasar/Seyfert/AGN phase. One can even make a rough estimate of how long this phase lasts. Only about 1% of galaxies are Seyfert/AGN, so any given galaxy ought to be in that phase for only about 1% of the age of the universe, i. e. perhaps 130 million years.
As noted above, there are various prominent characteristics that may or may not accompany the high luminosity of an AGN, including broad and narrow emission lines in the spectrum, strong radio emissions, and relativistic jets.
The broad emission lines are thought to originate in clouds of colder gas (under ~100 K) orbiting outside the accretion disk. Although such clouds emit little EM energy, they consist of atomic hydrogen, helium, and traces of heavier elements. Intense radiation coming from the disk will put these atoms in an energetically excited state. But when electrons drop back from higher energy levels, spectral lines at frequencies characteristic of each atomic species are emitted. Because the clouds are in rapid motion, the emission lines are broadened due to varying amounts of Doppler shifting of the spectral lines.
One would expect that such clouds should be present around all supermassive black holes. However, there are many AGNs, both quasars and Seyfert galaxies, in which broad emission lines are not observed. Seyferts were originally placed into one of two classes, according as broad lines were either present or absent. But now intermediate cases are known with only weaker broad lines, so intermediate types are recognized according to the prominence of broad line features.
The thinking now is that there is no actual difference between AGNs with and without broad lines. Instead, the full or partial absence of broad emission lines is ascribed to the degree by which the broad-line clouds are hidden within a thicker torus-shaped ring of even colder gas and dust that surrounds both the accretion disk and the inner clouds. Because of the thick toroidal shape, if our line of sight to the object is mostly face-on, the inner disk and the clouds will be visible. But if we see the object mostly edge-on, those features will be partially or fully hidden.
Narrow emission lines are seen in the spectra of most AGNs. The fact that the lines are narrower indicates that the gas they come from is not moving as rapidly as the gas responsible for broad lines. The type of lines and other evidence indicates that the source of the narrow line emissions is a large but diffuse corona of very hot, ionized gas, which can extend for many light years, surrounding all other parts of the AGN. In AGN that are close enough, the corona is large enough that its actual size can be measured directly. Much of the emissions from the corona is at far ultraviolet and X-ray wavelengths, showing that temperatures in the corona must be quite high.
Approximately 10% of AGNs, both Seyfert galaxies and quasars, are "radio loud" – that is, a source of strong radio frequency emissions. In fact, it was strong radio emissions from the first quasars to be recognized that sharply distinguished them from the normal stars they appeared to be at visible wavelengths. Now that many quasars can be recognized by the high redshift of their spectra – indicating very distant and hence very luminous sources – it turns out that only about 10% of quasars are radio loud.
Long baseline radio interferometry makes it possible to "see" the source of the radio emissions in some detail. The source is not spherically symmetrical, but instead takes the form of very long, narrow "jets", as seen in Centaurus A. Such jets can be hundreds of thousands of light years long. The evidence is that these jets consist of plasmas in which electrons near the central black hole can have relativistic velocities – with Lorentz factors of 104 or more. Electromagnetic emissions from jets often run all the way from radio up to X-rays.
The only plausible physical model for the jets requires very strong magnetic fields. These fields collimate the matter into narrow jets – which emanate in opposite directions from the central black hole – and accelerate the plasmas charged particles to extreme velocities. Relativistic electrons moving in a helical pattern around the jet axes are responsible for the radio emissions via synchrotron radiation.
Many of the details presented so far are based largely on theoretical models, even though astronomers have known of AGNs for over 50 years. Observational studies of active galaxies – quasars in particular – are difficult, since most of the objects are quite distant, and much of the action occurs in a volume of only ~100 cubic light years – impossible to resolve with existing technology. But observational evidence for some of the details is slowly accumulating. The research were now ready to discuss is an example.
The Hard X-Ray View of Reflection, Absorption, and the Disk-Jet Connection in the Radio-Loud AGN 3C 33
We present results from Suzaku and Swift observations of the nearby radio galaxy 3C 33, and investigate the nature of absorption, reflection, and jet production in this source. We model the 0.5-100 keV nuclear continuum with a power law that is transmitted either through one or more layers of pc-scale neutral material, or through a modestly ionized pc-scale obscurer. The standard signatures of reflection from a neutral accretion disk are absent in 3C 33: there is no evidence of a relativistically blurred Fe Kα emission line, and no Compton reflection hump above 10 keV. We find the upper limit to the neutral reflection fraction is R < 0.41 for an e-folding energy of 1 GeV. We observe a narrow, neutral Fe Kα line, which is likely to originate at least 2000 Rs from the black hole. We show that the weakness of reflection features in 3C 33 is consistent with two interpretations: either the inner accretion flow is highly ionized, or the black-hole spin configuration is retrograde with respect to the accreting material.
3C 33 (which means it is object number 33 in the Third Cambridge Catalogue of Radio Sources) has a redshift z=0.0597, which equates to a distance of about 800 million light years. 3C 33 is one of the brightest narrow-line radio galaxies (NLRGs). An NLRG is a radio-loud AGN in which the spectrum contains narrow width emission lines but there is little or no evidence of broadened spectral lines.
At visible and infrared wavelengths 3C 33 is nothing special to look at, but radio images show structures typical of radio galaxies, with pronounced lobes on both sides of the central object. (See here and here for images at various wavelengths.)
For an explanation of why 3C 33 is an interesting object of study, we need to go into a little more detail about what makes up the "hard" X-ray part of the spectrum of an AGN. This involves photons having energies from 1 keV to 120 keV.
The temperature of the plasma that makes up the corona is much higher than the temperature of the gas in the accretion disk, which is mostly un-ionized. Even the hottest parts of the accretion disk have their intensity peaks in the ultraviolet, with wavelengths of at most 10 nm, which implies temperatures of about 300,000 K by Wiens law. Hard X-rays with 12 keV photons are two orders of magnitude smaller in wavelength, implying temperatures around 30 million K. Quite a difference. So its not unreasonable to regard the gas in the accretion disk as "cold" – compared to the gas of the corona.
Its somewhat messy to describe what happens with very energetic radiation from the corona interacting with the less energetic radiation from the hottest (innermost) parts of the accretion disk. However, various simulation studies have investigated models where a hard X-ray spectrum is "reflected" from an opaque slab of relatively "cold" gas that mostly emits in the ultraviolet. High-energy photons can reflect off of lower energy electrons in the process of Compton scattering. The high-energy photons lose some of their energy in the process, while the lower-energy particles gain energy. The reverse can also happen: low-energy photons from the accretion disk can scatter off higher energy electrons and photons in the corona. In this process (inverse Compton scattering) the lower-energy photons gain energy.
The starting assumption is that the X-ray spectrum of the corona is approximated by a power law in which the distribution of number of photons of given energy is a power -α (with α>0) of the energy, i. e. N(E) ∝ E-α. Models with typical assumptions about the configuration of the accretion disk suggest that there should be a slight enhancement of number of photons at energies above 10 keV in the corona X-ray spectrum. This enhancement is referred to as the "Compton reflection bump".
The observational evidence is that this Compton bump is usually found in the X-ray spectra of radio-quiet AGNs. But radio-loud AGNs tend not to have this feature in the X-ray spectra, or have it only weakly. This suggests that there may be something different about the accretion disks of radio-loud AGNs – that is, AGN that also have a jet structure responsible for their radio emissions.
There is one other common feature in AGN X-ray spectra – an emission line from fluorescing iron (Fe) atoms around 6.4 keV. This is called the Fe Kα line. It is normally observed to be relativistically broadened, indicating that it arises from accretion disk reflection. Again, the Fe Kα line is commonly found in radio-quiet AGNs and not in radio-loud AGNs. This is a further indication of something different about the accretion disks of radio-loud AGNs.
3C 33 is a radio-loud AGN, so its a good candidate for closer investigation. However, theres an additional complication in all this in the radio-loud case. The jets radiate over the full EM spectrum, not just at radio frequencies. In particular, theres an X-ray component to the spectrum, and its especially strong at the base of the jets. If this part of the jets adds its contribution to the X-ray spectrum the shape of the spectrum will be changed so that the Compton bump (if any) is harder to distinguish.
As it happens, most of the radio galaxies previously studied have been of the broad line sort. Recall that this means we are seeing the galaxy more or less along the axis of the jet, so that the base of the jet and the surrounding accretion disk are not obscured by the outer torus of cold gas and dust. 3C 33, however, is a narrow line radio galaxy (NLRG). That means that the common axis of the jets and the accretion disk is at a large angle (more than ~60°) to our line of sight. Consequently we cant see the accretion disk or the base of the jets directly, due to the obscuring dust, and so broad emission lines arent visible.
Thats actually good, because it means unobscured X-ray emissions from the jets are relatively minor and would not make it difficult to detect a Compton bump and Fe Kα lines – if they were present. If there were a Compton bump, it would be due to photons reflected from the accretion disk and scattered to higher energies in the corona. Since the corona may be hundreds of light years in radius, it is not obscured.
Nevertheless, what this research has shown is that a Compton bump and significant Fe Kα fluorescence are not present in 3C 33. Therefore theres probably something different from the norm of AGNs about the accretion disk of 3C 33. And the most natural assumption is that difference is related to the jets.
What could the difference be? Another research team that has considered the issue of lack of Compton bump in radio-loud AGNs hypothesized that the hottest inner part of the accretion disk could be partially ionized. Therefore it would be semitransparent and not reflect photons strongly. Calculations showed that this was a viable hypothesis.
The team responsible for the present research has a different hypothesis: the strong magnetic fields that create the jets also force the inner part of the accretion disk farther away from the black hole – provided that the black hole itself is spinning in the opposite direction ("retrograde") from the accretion disk. So the research team suggests that the lack of Compton bump is possible evidence for opposing spins of black hole and accretion disk.
Even without effects due to the magnetic field, a retrograde spin of the black hole would cause the radius of the smallest stable circular orbit outside the black hole to be larger than in the prograde case. In other words, the material that would otherwise orbit closer to the black hole isnt there since it has to fall into the black hole. Since magnetic field lines cannot be anchored in a black hole, they must be attached to the accretion disk, and thus assume a different shape than they would if disk and black hole were spinning in the same direction.
At this point, there is no direct evidence for retrograde spin. The competing hypothesis of a semitransparent inner accretion disk isnt ruled out. Further study will be required to distinguish between the two hypotheses.
![]() | Evans, D., Reeves, J., Hardcastle, M., Kraft, R., Lee, J., & Virani, S. (2010). THE HARD X-RAY VIEW OF REFLECTION, ABSORPTION, AND THE DISK-JET CONNECTION IN THE RADIO-LOUD AGN 3C 33 The Astrophysical Journal, 710 (1), 859-868 DOI: 10.1088/0004-637X/710/1/859 |
Further reading:
Black hole spin may create jets that control galaxy (2/11/10)
Backward Black Holes Control Fate of Galaxies (2/12/10)
The Hard X-Ray View of Reflection, Absorption, and the Disk-Jet Connection in the Radio-Loud AGN 3C 33 – arXiv copy of research paper
Related articles:
Winds of Change: How Black Holes May Shape Galaxies (4/19/10)
Galactic black holes may be more massive than thought (6/8/09)
Black hole outflows from Centaurus A (2/6/09)
Evidence that quasars are powered by black holes (10/21/06)
The wind from a black hole (7/8/06)
Other resources
Black Hole Models for Active Galactic Nuclei – excellent technical introduction by Martin Rees
3CRR Atlas Home Page
NASA/IPAC Extragalactic Databse: NED
Subscribe to:
Post Comments (Atom)
No comments:
Post a Comment